Simon St.Laurent: September 2009 Archives
by Karl North
In Part One of this series, I noted that providing for the local food needs of urban populations requires a design that integrates three overlapping categories of production systems: urban agriculture systems (many small islands of gardening in the city center), peri-urban agriculture (larger production areas on the immediate periphery), and rural agriculture (feeder farms associated with village-size population clusters in the hinterland of the city but close enough to be satellite hamlets). In this month’s article, I will discuss four key issues that must be addressed in order to envision these three systems: fertility, energy, water, and pest control. But first, a word about the role of species diversity in addressing these issues.
In an energy descent environment, agriculture that incorporates the necessary diversity of species that are multifunctional — providing both ecological and other services and food — will gradually replace the current agriculture that substitutes external inputs to solve these problems.
Some of the most durable and productive low input farming systems in history are designed around animals that can accelerate the growth and conversion of plants to fertilizer. Because they are highly multifunctional, ruminant mammals rank highest among these. Beyond their manure production function, they can consume fibrous perennials unusable for human food. These perennials can grow on hill land too rocky or too erodable for food cropping. Used as work animals, ruminants multiply the energy input from human labor many times. They provide a source of concentrated protein food that can be conserved and stockpiled for winter consumption. They provide hides and fiber for clothing as well. Cattle, sheep, goats, alpacas, llamas and bison are ruminants that we can most easily use in agricultural systems in our environment.
A few other animals serve some of these functions and, properly integrated, often are found enhancing these systems. Pigs and poultry can do the hard labor of turning manure into compost, and can thrive by consuming unused and pest species as well as waste streams from farms and kitchens. They both can reduce a patch of weeds to bare ground ready for planting, and pigs will perform tillage as well. They will consume crop residues and garbage from food preparation, and convert it to fertilizer as well as their own production as food animals. Poultry will consume weeds and insect pests. Edible fish and other water animals like frogs and snails can perform the same functions in aquatic systems. This map of flows among components demonstrates the potential of integrated systems (Figure 1). Notice that the flows may go in both directions among all components:
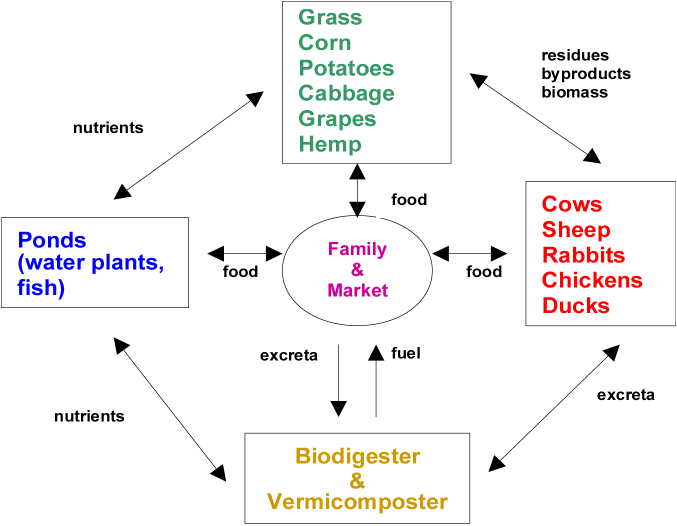
Figure 1. Dynamics of a hypothetical sustainable system
1. Soil Fertility
As energy descent deepens, two key fertility crutches of industrial agriculture will become cost-prohibitive. Synthetic nitrogen fertilizer production requires large quantities of energy. The decreasing quality of phosphate deposits is already driving up the price of phosphate fertilizer (up 700 percent in a recent 14-month period) and production is estimated to peak within 20 years.[1] Moreover, the affordability of most off-farm sources of fertility is derivative of cheap oil. But minerals essential to farm fertility can be recirculated within farms or at least within local food systems, and recirculation capacity will become essential to sustainable design.
On-farm recycling. Building high levels of soil organic matter (SOM) will be central to agroecosystem design because SOM is key to achieving not only fertility goals, but also healthy water and mineral cycles, maximal photosynthetic energy capture and use, and optimal biodiversity. Humid, temperate environment soils are exceptional in their ability to store organic matter. French scientist Andre Voisin demonstrated 50 years ago that pulsed grazing (explained below) on permanent pasture is the fastest soil organic matter building tool that farmers have, at least in temperate climates like ours.[2]
The structural element historically proven to work best in these environments is a grass/ruminant complex. This subsystem works on the principle that manure from a portion of the farm devoted to grazing animals will not only sustain the fertility of their forage land, but generate a surplus that will sustain a smaller acreage of annual crops (Figure 2). It can sustain fertility well enough to have generated numerous historical models around the world. The process was used in lowland northern Europe and New England before the industrial age.[3] Cuban research into its potential demonstrated the effective ratio of forage acreage to support cropland fertility to be 3:1 in that environment. In other words, the ruminant stock subsisting on three acres of forage produced enough manure to sustain both the fertility of the forage land and one acre of cropland. This conceptual model, adapted for environmental differences, provides a basis for system design here. Perhaps the most important design question for our purposes is the ratio of forage to cropland that is sustainable in our environment.
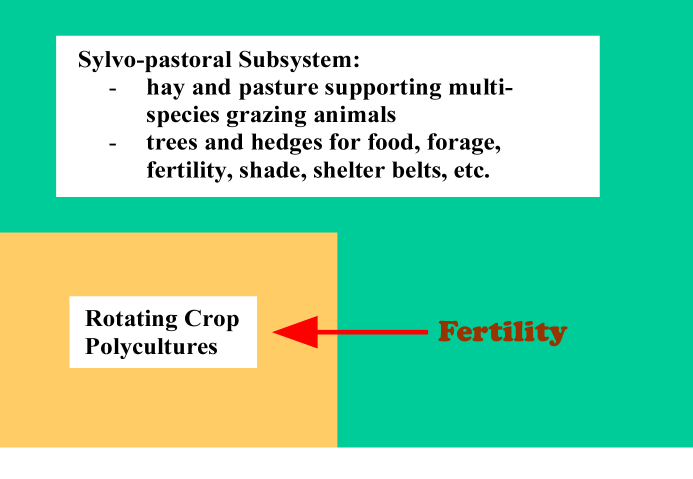
Figure 2. Fertility subsystem conceptual model
The full soil organic matter building process requires a design focus on three crucial areas of the agroecosystem:
Pasture management for a wide variety of productive, palatable perennial forages, kept in a vegetative state (high growth) by pulsed grazing (see below) throughout the growing season to maximize biomass production;
Manure storage in a deep litter bedding pack under cover during the cold season to maximize nutrient retention and livestock health;
Conversion of the bedding pack to compost at a proper C/N ratio during the warm season to maximize organic matter production, nutrient stabilization, and retention;
Field application of the compost during the warm season as well, to maximize efficient nutrient recycling to the soil.
Pulsed grazing is so important to the success of the soil building subsystem that it warrants an explanation in some detail. Pulsed grazing is a method of repeated grazing of paddocks in a pasture that controls stock density and timing of stock movement in and out of paddocks to maximize forage production over the growing season. This in turn maximizes manure production to build soil organic matter. Forage plants experience repeated pulses of growth and removal of biomass, both above and below ground, over the growing season. Key points :
Stock enter a paddock before forage leaves its vegetative stage and growth slows.
Stock leave a paddock while there is still sufficient forage leaf area to jump-start regrowth.
Grazing causes forage roots to die back, which adds soil organic matter from the dead root mass.
Stock return to the same paddock when leaf and root regrowth have fully recovered vigor and abiity to recover from another grazing.
Recycling from Human Communities. It should be clear from the integrated model (Figure 1) that solving the fertility problem must include repairing the broken nutrient cycle between human excreta and the land. If this seems an insurmountable challenge to modern urbanites, we need only recall from history that whole societies including large cities have managed excellent recycling of “night soil.” Among the numerous examples is China, where until the 1950s, 98% of the fertilizer used to grow food came from recycled and organic sources.[4] Relocalization of food production is necessary to reduce the cost of repairing the nutrient cycle. If Tompkins County exports milk products to NYC, what will it cost to return the nutrients in the exported milk to our farmland? In a more county-based food system, methods for recycling humanure and other food garbage that are appropriate to urban, peri-urban, and rural farming sites are more feasible, and will be discussed in the sections devoted to these production systems.
2. Energy Capture
Ancient sunlight in fast-depleting, finite sources (oil, gas, coal) presently supplies over 80% of the energy used in the industrial form of agriculture that produces most of the food consumed in the United States. Natural ecosystems consist of food chains supported entirely by current sunlight, so it is easy to design farming systems to work the same way, as was done through most of agricultural history. Solar energy that is accessible directly on farms comes in forms that are far less concentrated than the fossil fuels that we are used to. Therefore we need to design farms that can be productive on far less energy. The challenge is to capture solar energy in as many places as possible as it flows through the agroecosystem.
The carbon cycle is an important way solar energy flows through our world. All metabolic processes in agriculture and other biological systems release carbon to the atmosphere. Tillage that stimulates activity in the soil food web, animal and human digestion and composting are examples. But criticism of these processes as feeding greenhouse gas build-up is mistaken. Biomass conversion to food, fertilizer, or fuel is carbon-neutral over time because its emissions, unlike those of fossil fuels, are part of the biospheric carbon cycle. The important question here is how to manage the carbon cycle to maximize long-term levels of soil carbon sequestered as soil organic matter.
Animal Power. Currently (2009) people tend think of solar capture in terms of relatively high technologies like those that convert wind and sunlight to electricity. Working models exist of homesteads and even farms that are self-sufficient in electricity using small-scale equipment of this sort. However, most analyses of economic viability related to wind/solar electricity production at any scale are based on current costs in the manufacture and maintenance of these systems, all of which still rely on cheap oil. These analyses fail to account for already exponentially rising costs in raw materials and production of the equipment. All production costs of such technologies will rise in parallel with sharply increasing energy costs as the fossil fuel era declines. Like oil, many raw materials used in these technologies are finite resources already on the downside of their historical production curve; they will become unaffordable for many uses in the future. In sum, the window of opportunity that makes these alternative energy technologies approach economic viability now may close in the future as costs begin to rise more sharply. A 10kw wind-electric rig that can power a small farm costs about $70,000, and is usually economically unfeasible even today without subsidies. What will it cost after 15 years of rising manufacturing costs? What will it cost to replace it after its 20-30 year lifetime?
However, there are ways of powering farm production that are more reliably sustainable. Just as the same breeze or brook flowing through a community might be tapped at a number of points for wind or hydropower to run a mill or pump water, solar energy can be captured to produce food or fuel by inserting species appropriately into the farm food chain. Apart from wind and flowing water, solar energy enters the farm ecosystem via photosynthesis in green plants, and flows through the system as one species feeds on another. Large herbivores tap immediately into this chain by feeding on plants that are too fibrous for food use. While they may produce food and fertility as previously described, they will do double duty as work animals in the future, thus replacing no longer affordable fossil-fueled machine labor.
Fields that grow the forages that support work animals and other grazing and foraging species will not compete with cropland. On the contrary, forage fields will provide an essential ecological service as the permanent cover necessary to sustain soil health on all sloping land. Present hillside cropland is always eroding and will be revealed as unsustainable when the crutch of cheap synthetic fertilizer is no longer available. This means that land use plans in hill country like ours will need to include a mosaic of hillside forage land and relatively flat cropland. Unless terraced, the hillsides will be most erosion-free and productive when planned to mimic natural tree-dotted savannas, as hay/pasture that includes fruit and nut orchards, for example. The trees themselves will be multi-functional, producing food or forage, improving the cycling of soil nutrients, providing windbreaks, and shading the grazing animals.[5]
Integrated as described here, draft animals like oxen, mules, and horses will optimize the health and productivity of the agroecosystem.
Biofuels. Energy for winter heating and for cooking is almost as important as food production for survival in these latitudes. As much as possible of that energy should come directly from the sun, as in passive solar designs for both heating and cooking. But rural land use will need to reflect increasing local dependence on firewood for the rest. Sustainable forest management and harvest will again become a significant share of rural agricultural production, but serving local urban and village communities not faraway paper mills. Forest conservation and reforestation should start with places that need to be forested for additional reasons, like ridge tops that protect water catchments, and hedgerows that serve as shelterbelts and browse for livestock.
Production of most other biofuels at any significant scale has been criticized as unsustainable on many counts. One that may prove sustainable is small-scale biogas generation on farms, because it extracts methane from some of the farm’s normal manure production before it continues in the farm’s nutrient cycling loop, as in Figure 1. Most attempts at biogas generation on US farms have been large-scale, high-technology projects aimed at fixing the pollution problem caused by industrial scale dairy farming. So far, farmer adoption of the expensive and complex equipment has been poor, despite subsidies. Meanwhile, small scale biogas generators aimed at producing light and cooking fuel in Third World peasant communities have proliferated, because they cost as little as $30.[6] Biogas production requires no separate biofuel crop that might compete with food production, or inefficient distillation process. For these reasons biogas production at an appropriate scale merits consideration as a way of capturing solar energy as methane fuel for limited use on farms and perhaps even surrounding communities.
3. Water Capture and Use
We live in a climate that is wet yet subject to droughts during the growing season. High productivity food production requires a constant water supply to cover these gaps. Maximizing productivity in the small areas devoted to urban agriculture is especially important, because of their high value in a relocalized food system. Sufficient water falls on urban areas and needs to be conserved there. Barrels can catch only a fraction of roof runoff, and will not be enough for the irrigation needs of a successful urban and peri- urban agriculture. Small water catchment ponds must become a normal part of both the public and residential urban landscape. Pavement runoff will need to be directed to the larger ponds, which might be located in parks and community gardens.
Rural agriculture will need more extensive water capture plans to hold and use water for farms and whole watersheds. Such a system should be gravity feed system, in order to avoid the increasingly high cost of pumping. An example is the keyline plan that traps some surface water in upper fields and directs the excess into strategically located irrigation ponds.[7]
Our irrigation needs in New York may be intermittent but still will require a lot of pipe and other delivery hardware when scaled up to cover all food production land. Rising costs of current irrigation delivery systems may become a limiting factor, forcing the invention of ones that use cheaper materials. This has been the experience in Cuba, whose year-round agriculture is heavily dependent on irrigation. Cuba’s artificially triggered “peak oil” experience has been a bellwether and a source of lessons for the rest of the world.
Ponds will be needed to serve numerous purposes, as in Figure 1. Basins to process biodigester outflow and other organic liquid waste can grow algae and duckweed for animal feed, and then feed the cleansed water into ponds for fish and other aquaculture, as in Figure 3. They will attract aquatic life including species useful for garden pest control, and enhance human quality of life as they beautify places and improve microclimates.
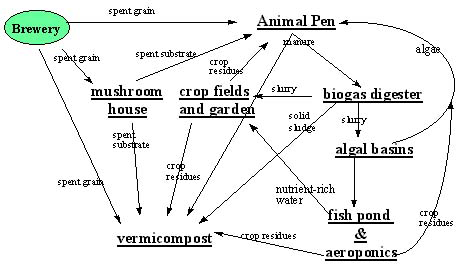
Figure 3. Facilities for bioconversion using the UNU/IAS integrated biosystem at Montfort Boys Town, Suva, Fiji
Wetlands abound in New York and are among the most productive natural ecosystems. Because of their natural potential, they can be harnessed for highly productive agricultural use yet be managed to retain much of their natural function. Historical and contemporary models include wetland systems that fed older civilizations from the Aztecs to the Incas in Latin America, as well as many parts of Southeast Asia today. Typically, as in the Aztecan systems known as chinampas, farmers cut canals through the wetland and use the soil to create beds raised above the water level for agricultural use. The canal system is designed to allow the water control that keeps the raised beds well watered without being subject to undesirable flooding. Because of the ubiquitous water, these wetlands are highly productive as both agricultural and aquacultural systems. They produce so much biomass that they tend to maintain their own fertility, dredged from the decomposing detritus in canal bottoms.
One such wetland, adapted from lowland English agriculture, became the core of a highly sustainable agricultural system that supported the population of colonial Concord, Massachusetts for many generations.[8] The Great Meadow that traversed the village and all other nearby riverine flood plains was a swamp commons that was first flooded to deposit silt, then partly drained and reserved for pasture and hay as it dried out during the growing season. As in parts of Europe, these well-watered riverine meadows produced enough livestock feed, livestock, and manure to sustain the fertility of the adjacent dry lands devoted to tillage agriculture. Figure 4 shows that already by 1650 careful allocation of land use had taken place on a functional level to sustain the whole system. Historical models like these suggest that we will want to regard modified wetlands as an important agricultural asset in the energy descent era.
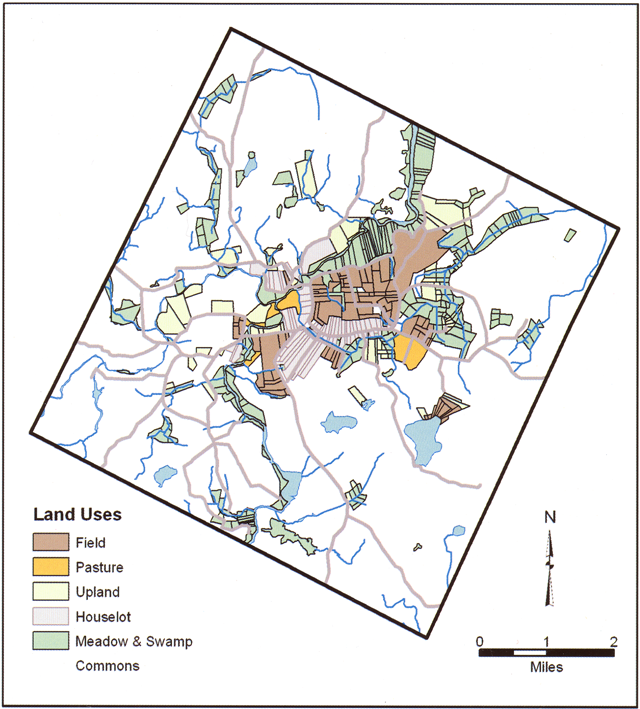
Figure 4. Concord, Massachusetts, 1652. From The Great Meadow: Farmers and the Land in Colonial Concord.
4. Pest Control
From a systems perspective, pest problems are “structural,” hence best addressed by system design rather than treatment with pesticides. In this section I will summarize two main strategies addressed in order of importance: a focus on the food species themselves, and then the layout of the physical and biological environment as it affects these food species.
Much as health care in humans requires preventive medicine, we must grow healthy plant and animal species as a first step in pest control. A primary structural problem is the genetic industrialization of most agricultural plant and animal species, which was gradually achieved in modern times by breeding processes that prioritized productivity and short-term profit over other genetic traits, like hardiness. Moreover, relying on pesticides, even “natural” ones, to protect these weakened subspecies inevitably fails over time because pests gradually adapt to conditions and treatments that become heavy- handed and routine. An example is parasite resistance in sheep, which has been neglected and lost. The resulting industrial breeds must be medicated so often that the parasites are gradually becoming immune to most medications. To be sustainable, food production systems will need to return to varieties and breeds that, while sometimes less productive, have more genetic defenses. By genetic selection farmers can rebuild hardiness in industrial breeds as well.
The design of alternative environments uses three general strategies of pest control: luring or driving them away with trap or repellent species or physical barriers; creating species and habitats that attract “beneficials,” species that prey on pests; and continually altering the environment with crop and animal rotations that shift them away from pests.
This last strategy points up a characteristic of the natural world that needs to be taken into account: it is always evolving. In the long run this means that pest control strategies can never be permanent, but must always be evolving to stay a step ahead of pests as the latter adapt to current controls. The downfall of industrial pest controls is their heavy- handed strategy of total pest elimination and routine medication. Ironically this creates the environments most conducive to genetic evolution in pest organisms toward immunity from controls.
Recourse to medicinals and other treatments is a strategy of last resort, indicating a design failure in the production system, which must be addressed.
Conclusion
From the foregoing it seems clear that life after fossil fuels will demand much reorganization of food production. To create a local agriculture that feeds the county, the map of rural and urban land use will change dramatically. In the countryside, wetlands and floodplains, hillsides, flatlands, and woodlands will have specific uses designed to maximize while sustaining the productivity of whole agroecosystems. Essential rural land use components might be:
Hillsides in forage land sufficient to support cropland fertility.
Flatlands in crop rotations.
Wetlands and floodplains development and water management for high forage or crop production.
Sufficient forest for county firewood and basic construction needs, managed for maximum regenerative capacity, which requires fencing out livestock. Woodland regenerative capacity equaling 1 cord/acre/year is a common rule of thumb.
Many uses of city land will no longer be economical in the coming years. Land will need to be converted to food production and its supporting functions, like composting and water conservation. Prime candidates for conversion are the commercial strips now inhabited by national corporate chain stores. Private and public parking lots, which energy descent writer William Kunstler sees as soon-to-be-dysfunctional “missing teeth in the urban fabric,” are another example. During Cuba’s artificially triggered encounter with “peak oil,” public interest dictated that a better use of resources was to raze ageing buildings to create urban garden space, rather than to restore them.
In the integrated system approach described here, the functions of plants and animals will undergo marked changes. The functions of many species to facilitate tight nutrient cycling, labor, and other services that underpin the health of the whole agroecosystem, will become more important. In the case of some animals, these functions will become primary, and food production will become a secondary function, with numbers of animals on farms directed to their primary functions. The result will be a general production system model that aims for maximum sustainability, remains within the carrying capacity of the natural resource base, and within that framework, feeds the maximum number of people per acre of land used.
Notes
[1] Peak Phosphorus: The Sequel to Peak Oil http://phosphorusfutures.net/index.php?option=com_content&task=view&id=16&Itemid=30
[2] Voisin, André. Grass Productivity, 1959 (English translation in 1988). Island Publishers, Washington, D.C., U.S.A.
[3] Donahue, Brian. 2004. The Great Meadow: Farmers and the Land in Colonial Concord. New Haven:Yale University Press.
[4] http://www.fairviewgardens.org/pub_next_frontier.html
[5] North, Karl. 2008. Optimizing Nutrient Cycles with Trees in Pasture Fields. LEISA Magazine, 24 (2), March 2008. http://www.ileia.org/index.php?url=magazine-list.tpl&p[source]=ILEIA
[6] Preston, T.R. 2005. Biodigesters in Ecological Farming Systems. LEISA Magazine, 21 (1), March 2005. Also: http://www.ruralcostarica.com/biodigester.html
[7] http://www.keyline.com.au/ad1ans.htm
[8] Donahue, op. cit.